Quantum Dots by Nature
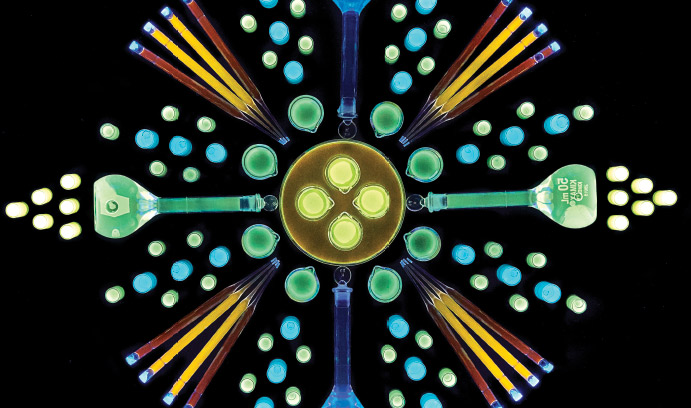
Quantum dots are used in transistors, solar cells, LEDs, lasers and medical imaging. Current industrial production processes are time-consuming and environmentally unfriendly. Three Lehigh researchers have found a better, more natural approach to the production of quantum dots.
Lehigh researchers have successfully demonstrated the first precisely controlled, biological way to manufacture quantum dots. This one-step method starts with engineered bacterial cells in a simple, aqueous solution and ends with functional semiconducting nanoparticles, all without resorting to high temperatures and toxic chemicals.
Born of an ideal collaboration among three Lehigh faculty researchers, this elegant technique has great potential for green manufacturing of quantum dots (QDs), which are used in transistors, solar cells, LEDs, lasers and medical imaging. Current industrial processes to produce them are messy, requiring toxic solvents, heat and high pressure.
Bryan Berger, associate professor of chemical and biomolecular engineering and bioengineering, wondered in 2012 whether the bacterial strain Stenotrophomonas maltophilia could be manipulated to generate QDs on command, since it had previously been known to exhibit heavy metal resistance.
Berger teamed up with colleagues Steve McIntosh, associate professor of chemical and biomolecular engineering and member of Lehigh’s Class of ’61; Chris Kiely, the Harold B. Chambers Senior Professor of Materials Science and Engineering; Robert Skibbens, professor of biological sciences; and Ivan Korendovych, assistant professor of chemistry at Syracuse University. Together they won a $2 million grant in 2013 from NSF’s Division of Emerging Frontiers in Research and Innovation (EFRI) to prove the production of cadmium sulfide QDs using an engineered form of Stenotrophomonas. They tailored the bacteria to grow nanocrystalline metal sulfides including QDs, building on earlier research, which was funded by Lehigh’s Faculty Innovation Grant (FIG) and Collaborative Research Opportunity Grant (CORE) programs.
“The beauty of a biological approach is that it cuts down on the production needs, environmental burden and production time quite a lot,” says Berger. Industrial processes take many hours to grow the nanocrystals, which then need to undergo additional processing and purifying steps. On the other hand, biosynthesis takes minutes to hours maximum to make the full range of quantum dot sizes (about 2 to 3 nanometers) in a continuous, environmentally friendly process at ambient conditions in water that needs no post-processing steps to harvest the final, water-soluble product. Because bacterial cells are much larger than the nanocrystals, researchers simply use a centrifuge to pull the cells away from the QDs in the solution.
More recently, the researchers have branched into creating lead sulfide QDs and are working on oxide-based materials, widening the range of QD practical applications. Their underlying technique allows them to control the particle size to fractions of a nanometer, an essential function, since particle size determines the QD’s optical and electronic properties.
Cell-based growth needs only basic equipment in a typical biochemistry wet lab setting. Using a process called directed evolution, researchers altered the bacteria so they would selectively produce quantum dots. Housed in a beaker containing water, cadmium and sulfur precursors, and minimum levels of carbon and nitrogen, the cells forgo most normal biological functions. They build quantum dots by sequestering metal ions from their environment, generating a reactive sulfur source and controlling the resultant structure to form a crystal.
Perfecting the methodology to structurally analyze individual nanoparticles required a highly sophisticated Scanning Transmission Electron Microscope (STEM). Lehigh’s Electron Microscopy and Nanofabrication Facility was able to provide a $4.5 million state-of-the-art instrument that allowed the researchers to examine the structure and composition of each QD, which is only composed of tens to hundreds of atoms.
“Even with this new microscope, we’re pushing the limits of what can be done,” says Kiely. The instrument scans an ultra-fine electron beam across a field of QDs. The atoms block the electrons in the beam, producing a kind of shadow image on a fluorescent screen, akin to the way an object blocking light produces a shadow on the wall. A digital camera records the highly magnified atomic resolution image of the nanocrystal for analysis.
An early challenge for the team was figuring out how to separate QDs from the biological material that surrounded and obscured them. “It was a learning process to clean off the organic residues. Once you get rid of that, you begin to see the true structure of the material,” says Kiely. “It took many, many months of frustration for our dedicated team of students (Zhou Yang, Leah Spangler, Chris Curran, Robert Dunleavy and Li Lu) to figure out exactly how to do this. Now that we have a much better understanding, we’re starting to accelerate our progress of new materials discovery.”
The researchers’ work was featured on the cover of the July 2015 issue of Green Chemistry. The article suggests that because a single enzyme in the bacteria is responsible for QD generation, the current cell-based model could be scrapped entirely in the future. QDs could be generated with the same enzyme synthesized from yeast or some other easily manipulated bacteria, says Berger. “We have fairly strong evidence for a cell-free process. It’s more cost-effective to use just a purified enzyme,” he says. The team is pursuing an extracellular approach and is poised to scale up its laboratory success into a future manufacturing enterprise.
The researchers hope to establish a manufacturing company that makes inexpensive QDs in an eco-friendly manner. Conventional manufacturing costs $1,000 to $10,000 per gram. A biomanufacturing technique could potentially slash the price by at least a factor of 10, and the team estimates yields on the order of grams per liter from each batch culture, says McIntosh.
Taking a long view, the three colleagues hope that their method could lead to a plethora of future QD applications, such as greener manufacturing of methanol, an eco-friendly fuel that could be used for cars, heating appliances and electricity generation. Water purification and metal recycling are two other possible uses for this technology, because the enzyme makes QDs by isolating heavy metals from water.
“We also want to create many different types of functional materials and make large-scale functional materials as well as individual quantum dots,” says McIntosh. He imagines developing a process by which quantum dots arrange themselves into macrostructures, the way nature grows a mollusk shell out of individual inorganic nanoparticles. “If we someday get to macroscale, if we’re able to make more of the material and control how it’s structured while maintaining its core functionality, we could potentially get a solar cell to assemble itself with quantum dots. That would be incredible.”
Bryan Berger’s research is focused on problems at the interface of biophysics and engineering. He and his team work extensively with clinicians and biomedical scientists. Berger received his Ph.D. in chemical engineering from the University of Delaware and is a recipient of the NSF Faculty Early Career Development Award (CAREER).
Steve McIntosh pursues research areas that are interdisciplinary in nature and encompass the fields of catalysis, electrochemistry, materials chemistry and reaction engineering. He received his Ph.D. from the University of Pennsylvania and is a recipient of the NSF CAREER Award.
Chris Kiely applies advanced analytical electron microscopy techniques in the arenas of catalysis and nanotechnology. He serves as director of Lehigh’s Electron Microscopy and Nanofabrication Facility. Kiely received his Ph.D. from Bristol University.
Story by Manasee Wagh
Photo by Christa Neu
Posted on: